- 1Center for Circadian Biology, University of California, San Diego, La Jolla, CA, United States
- 2Oregon Institute of Occupational Health Sciences, Oregon Health and Science University, Portland, OR, United States
- 3Department of Psychology, University of California, San Diego, San Diego, CA, United States
- 4Departments of Psychiatry and Neuroscience, Uniformed Services University of the Health Sciences, Bethesda, MD, United States
In this review, we discuss the remarkable potency and potential applications of a form of light that is often overlooked in a circadian context: naturalistic levels of dim light at night (nLAN), equivalent to intensities produced by the moon and stars. It is often assumed that such low levels of light do not produce circadian responses typically associated with brighter light levels. A solid understanding of the impacts of very low light levels is complicated further by the broad use of the somewhat ambiguous term “dim light,” which has been used to describe light levels ranging seven orders of magnitude. Here, we lay out the argument that nLAN exerts potent circadian effects on numerous mammalian species, and that given conservation of anatomy and function, the efficacy of light in this range in humans warrants further investigation. We also provide recommendations for the field of chronobiological research, including minimum requirements for the measurement and reporting of light, standardization of terminology (specifically as it pertains to “dim” light), and ideas for reconsidering old data and designing new studies.
Introduction
Light has a profound influence on the biological health of mammals, including humans. In addition to illuminating the environment for vision (i.e., image forming), light also regulates non-image forming processes, such as the circadian timing system, neuroendocrine fluctuations, and acute alerting effects, just to name a few (1, 2). All of the non-image forming effects of light studied to date appear to be most sensitive to short-wavelength light and at intensities greater than what is required for visual illumination (3). As current architectural lighting strategies have been developed around the human visual system, most do not fully support biological health and well-being. Yet, the timing of photic delivery is also of utmost importance: one major non-image forming function of light is to synchronize internal biological rhythms—orchestrated by a master pacemaker in the suprachiasmatic nucleus (SCN) in the hypothalamus—to environmental light-dark cycles. This process is called entrainment. To provide a daily photic temporal cue in lieu of exposure to the solar light dark cycle, a biologically potent light stimulus should be used to signal daytime (4), whereas a relatively less potent stimulus (dimmer and depleted in shorter wavelengths) should be utilized during the evening and night. It has been proposed that the current suboptimal patterns of photic exposure, including insufficient light during the day and excessive light at night, are at least partly responsible for a variety of negative health and safety consequences (5, 6).
Interestingly, much of the seminal work characterizing non-image forming responses to light has taken place during the biological night, yet the focus of lighting countermeasures in humans has been on optimizing photic exposure during the day. By far, the majority of these studies assesses the responses elicited by exposure to a short duration pulse of light (typically <2 h) against a background of complete darkness or dim light. Using this approach, investigators have operationally defined the lower limits of light sensitivity with respect to these biological responses to acute light pulses. A growing body of literature, however, demonstrates that light far below these putative thresholds for circadian sensitivity nevertheless exerts potent biological effects in non-human mammals, particularly if the illumination is present over several hours (7, 8).
Compared to complete darkness (to the extent that this can be feasibly created in the laboratory), dim illumination in the range approximately of moonlight and starlight markedly alters the fundamental properties of circadian rhythms in model organisms. Specifically, the addition of a relatively small number of photons throughout the biological night enhances the flexibility of behavioral entrainment by traditionally bright lighting regimens. These rarely recognized, and highly potent actions of very low light doses have been particularly well-documented in nocturnal mammals. Given the broad conservation of circadian (neuro)biology (e.g., molecular feedback loops, brain structures) and function (e.g., phase-shifting, light sensitivity) across taxa and ecological niche (see below for more detail), it is critical to ask whether comparable effects could be elicited in humans. If so, dim light treatments would represent a novel and more efficient approach for correcting or preventing circadian disturbances, particularly in more extreme cases, such as is common with jet lag and shift work. This review first aims to clarify terminology on different definitions of “dim light” and summarize the effects of very dim light in model systems. We limit the scope of our review to circadian research in mammalian organisms because of the focus on potential translational value for human circadian studies. Next, we discuss why the biological potency of very low levels of dim light should be considered and examined in humans. Although some reports showing effects of lunar cycles on human sleep exist (9–12), there are few experiments examining the effects of comparable dim light levels on circadian endpoints in humans. Therefore, we identify a range of opportunities for future human work and include recommendations for the field going forward.
Different Levels and Definitions of Dim Light and Dark
Light levels may be reported in photometric (e.g., lux), radiometric (e.g., μW/cm2 or photons/cm2/s) and the emergent melanopic EDI (also in lux). If sufficient information is provided, which must include the spectral power distribution (SPD), these measures can all be converted into each other (13, 14). Because of very different scales, however, comparing across metrics may not always be intuitive, especially with polychromatic light. Throughout this review, we prioritize presenting measures as reported in the original work, but have converted to melanopic lux when possible and when useful for comparison.
Across the circadian literature, studies have used dim light for different purposes and defined a wide range of light levels as “dim” (Figure 1). These studies can generally be divided into three categories. The first group aims to eliminate any form of temporal information by exposing subjects to equal amounts of light throughout the night and day. It is worth noting that despite constant levels of environmental light, orientation of the head as well as opening and closing of the eyes inevitably leads to varied photic exposure patterns. In humans, dim constant light is more commonly used to study intrinsic clock properties that are not directly driven (or masked) by light (20).
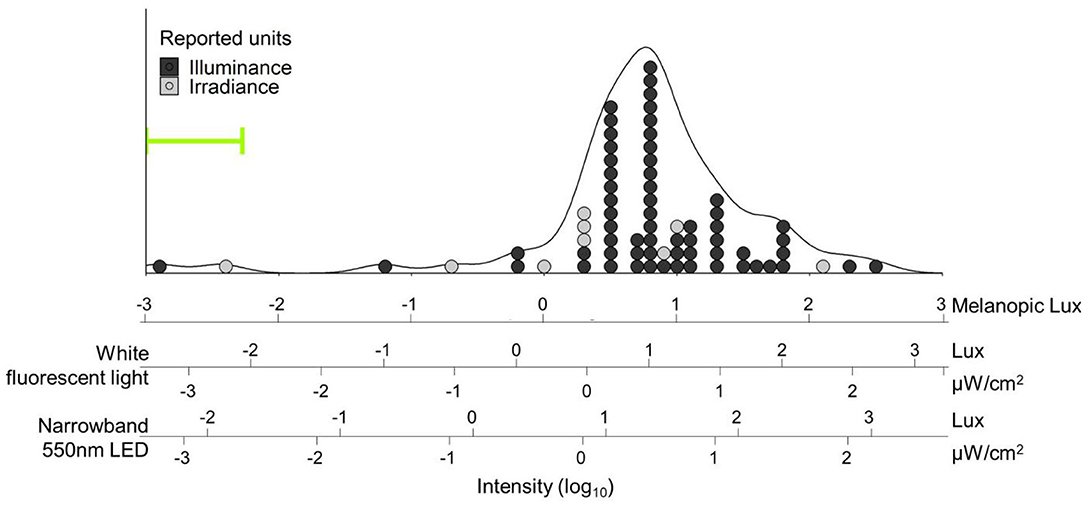
Figure 1. Distribution of light intensities referred to as “dim light” in human circadian research papers. Light intensities were converted into melanopic lux [using the Lucas toolbox 2014 (13)] and plotted on log units. Additional scales with white fluorescent light and narrowband green (550 ± 23 nm) light are provided and aligned for equivalent biological potency [e.g., “10 lux” (1.5 μW/cm2) narrowband green LED light has the same biological potency as 4.7 lux while fluorescent light: 2.92 melanopic lux]. Fifty-five percent of the papers (39/71) did not report any spectral or color information about their light, so SPD for fluorescent white light was assumed for conversion. Overall density is represented by the black line. Individual papers are plotted as dots, and color coded for the reported unit type; 62/71 (87%) reported light intensities in lux alone. Both mode (tallest stack) and median intensities reported as “dim light” in our search were 10 lux fluorescent white light (6.2 melanopic lux) – bright enough for phase shifting (15), entrainment (16), or melatonin suppression (17) in humans. The three papers below 0.1 melanopic lux all reported the use of red dim light; all other papers used either white light or did not report color information. Range of intensities used in Gorman lab rodent studies is indicated by the green bracket, and extends to lower values outside the bounds of the figure (see Table 1). Search strategy: A pubmed search was performed on October 23, 2020 with search terms: “human circadian dim light.” Results were filtered for “2,000-present” and “Journal Article,” which resulted in a total of 509 articles, and sorted for “Best Match.” The first 100 results were downloaded and screened for light intensity information. In total, 29 papers were excluded because they were either review papers (n = 11), used non-human subjects (n = 13), or reported neither intensity nor source (n = 5). From each paper the brightest light referred to as “dim light” was recorded. If the paper reported a range (e.g., 1–5 lux, or <5 lux), the highest value within the range was used. If multiple units were reported (e.g., Lux and μW/cm2) irradiance was used over illuminance.
The second body of work aims to mimic urban levels of artificial light at night to study its effects on sleep or health (21, 22). These studies usually focus on the ramifications of our modern lifestyle and the ubiquity of artificial light on natural processes, such as exposure to electronic devices, indoor LED lighting, or light derived from artificial light sources outside, such as street lights. Thus, such studies typically use 5–30 lux during the “night,” as opposed to near darkness. These protocols lead to all kinds of health decrements, including loss of sleep and metabolic syndrome in both rodents [reviewed in (23)] and humans [reviewed in (24)].
The last group of studies uses much lower levels of light (commonly 0.01–0.1 lux) that are more consistent with natural light at night (nLAN) cast by the moon or stars (9–12, 25–38). In contrast to the relatively brighter light used in constant light or studies of urban nighttime light, nLAN seemingly does not induce circadian health disruption, though data are limited. It does, however, have marked effects on circadian organization of behavior, despite being reported as below threshold for phase shifting or melatonin suppression. These changes in circadian behavior, which generally indicate a higher degree of circadian flexibility, have so far been primarily studied in rodents, but yield strong translational potential in aiding the adjustment of the human circadian clock to either rapid travel across multiple time zones or irregular schedules such as in shiftwork.
To illustrate the wide range of light levels referred to as “dim light” by circadian researchers, we performed a literature review and gathered light parameters summarized in Figure 1 (see legend for search methodology). When reviewing these articles, we noticed that, often, investigators reported extensive detail about the bright light stimuli employed in their studies but did not quantify or qualify the “dim light.” Specifically, 39/71 (55%) only reported “dim light” levels in lux (only relevant for the image-forming system), without any information on spectral quality or source; a further 5/76 (6%) were excluded from our analyses because they did not even report intensity.
Because absolute darkness in the laboratory often cannot be feasibly achieved or measured with any confidence, it is difficult to define precisely where the “threshold” is between (very) dim light and darkness. Walls, doors and ceilings are rarely completely light-tight, and equipment in or near animal housing may also generate light. The QA-4 modules in the VitalView data collection system commonly used for circadian monitoring, for example, have red indicator lights that fluctuate when an activity signal is received and can produce feedback (39). Moreover, investigators may intentionally put in place constant, low levels of illumination for practical reasons, such as animal husbandry (6), sometimes without reporting. Careful exclusion or attenuation of all such light sources can, of course, diminish light exposure.
It is our impression that many circadian researchers share the assumption that (very) dim light is biologically equivalent to actual darkness. Such beliefs might be founded on carefully executed experiments demonstrating minimal light levels that are required to induce phase shifts or melatonin suppression (40–42). As we will lay out in this review, however, there is a multitude of evidence, within the circadian literature, as well as from other fields, that light well below 1 lux can have dramatic effects on circadian oscillators or their outputs, challenging these common assumptions. By summarizing these (perhaps unintentionally) overlooked effects of very dim light levels within circadian research, we hope to provide a framework to aid researchers in designing and reporting on their experimental lighting conditions.
Historical View of nLAN Studies
Laboratory studies cannot escape trade-offs between simplicity and experimental control on one hand and real-word validity on the other. In the domain of circadian entrainment, this trade-off has been operationally resolved in favor of exposure to an alternation between just two photic conditions, each of unvarying spectrum, intensity and geometry, to represent “day” and “night.” Twilight transitions, lunar cycles, and variability due to cloud cover are just a few examples that reflect naturally-occurring changes in intensity and spectrum that are rarely simulated, although each of these photic dimensions may be highly relevant to circadian timing systems of particular organisms, and it could be adaptive for such organisms to be sensitive to them (43). The work in the 1960's of zoologist JL Kavanau (44–46) on rest/activity cycles of mammals provided myriad demonstrations of the behavioral importance of additional domains of photic stimuli. For example, running speed, perhaps a reflection of motivation, was sensitive to light intensity during twilight; rodents of various genera operantly manipulated light intensity in relation to their endogenous rest/activity cycles; and animals expressed reliable (but non-homogenous) orientation preferences toward light sources, such as always running toward a dim night-time light source, conceptualized by the investigator as a simulated moon. In short, there emerged a rich ecology of environmental lighting that did not, for whatever historical reason, strongly imprint on the emergent field of chronobiology, though the zoological tradition remains important (30, 37, 47).
Serendipitous Discovery of nLAN Effects in an Experimental Setting
It came as a great surprise when we accidentally “rediscovered” the fundamental importance of dim illumination on circadian entrainment, when we thought we knew it to be of little consequence. In preparing for a time series collection of Syrian hamster (Mesocricetus auratus) brains for a study of SCN rhythmicity in a paradigm termed “bifurcation,” we discovered notable and nearly categorical entrainment differences between the first and second cohort of animals. We had thought that each set of 20 hamsters was exposed to identical conditions, which included a very dim green light that was continuously illuminated—day and night—to aid in working with animals at night. These lights (see Table 1 for intensities) had been incorporated after thorough (but perhaps not thorough enough) consultation of the literature suggested their inefficacy (e.g., thresholds for melatonin suppression and phase shifting) and some further experimental validations that they did not induce classic circadian responses. Confronted by the statistically improbable result that chance alone caused animals 1–20 to respond one way and animals 21–40 another, we looked for other explanations, only to discover that the electrical power for dim nocturnal illumination had been disrupted in half of the animals. Although we approached this explanation with skepticism, a de novo-test confirmed a potent entrainment role of this very dim light source (48). Since that time, we have come to appreciate that the effects of nLAN are more generalizable than we initially imagined.
Demonstrations of a potent circadian role for nLAN both preceded and ran parallel to our work [reviewed in (30, 37, 47)]. Working with bats, for example, Erkert demonstrated systematic variation in circadian entrainment parameters with variations in light intensity in the range of starlight (32–35, 49). Readers interested in the ecological breadth of the biological effects of nighttime light could probably not do better than to consult a comprehensive resource, such as that from Rich and Longcore (47), or this recent review (50). But in the circadian domain specifically, our lab has accrued a critical mass of studies contrasting fundamental properties of circadian rhythms in animals under dimly illuminated vs. conditions without detectable light. We present a synthesis here that is not easily derived from reading the publications as they individually appeared.
Range of Parameters Used in our Lab Over the Years
Over the years, we have used a variety of nLAN sources. Our initial studies assessed the efficacy of a single green narrowband LED (560 nm, full width at half maximum of 23 nm) mounted on the wall of a light-tight enclosure containing a single opaque plastic cage that provided some diffusion. Other studies employed panelescent LED nightlights ~1 m from free-standing caging racks, with animals housed in clear cages. Most of our studies have used a single green LED mounted in a standard position ~10–30 cm from the orthogonally oriented running wheel in transparent cages (Table 1).
Unlike highly controlled human studies that can employ Ganzfeld domes, pharmacological pupil dilation etc., the animal housing environment allows ample opportunity for variation in effective light exposure. Although in various enclosures we have taken pains to minimize variability in light exposure via diffusers, positioning of cages, etc., conventionally, we have reported light intensity at the brightest location in the cage. But as intensity falls off by the inverse square law, it can vary considerably within the cage. Moreover, animals oriented directly toward the light [as in Kavanau's rodents (44)] might, depending on the experimental setup, be sampling several orders of magnitude more light than animals with their gaze diverted from the point source. Conversely, effective illumination of the retina could be attenuated with pupillary constriction.
In short, it is not yet possible to define with any precision the range of parameters of nLAN that produce a substantial effect on rodent rest/activity cycles, but the sources and configurations used do not appear to be near the limits of an operating range. Effects appear to be robust with respect to some variation in caging configuration and light orientation, although systematic assessments of these factors have not been completed. Finally, the narrowband green light is likely to activate multiple photoreceptor mechanisms. In limited studies, white light proved also to have efficacy, so we have little reason to believe that narrowband stimulation is a requirement, although this remains to be thoroughly assessed.
Despite a variety of enclosures as well as light sources and intensities (see Table 1) used throughout two decades of experiments to investigate the effect of simulated natural night light levels, the nLAN conditions were always deliberately contrasted with darkness, created by careful exclusion or attenuation of all light sources. As mentioned above, typical laboratory conditions do not achieve complete darkness. In our lab, we made every effort to exclude light to approximate complete darkness. Even after covering all equipment LEDs with black electrical tape and blocking all visible light coming into the room through doors or ventilation, however, our dark conditions may not be absolute. Nevertheless, they were always below the limits of detection (using an IL1700 radiometer; International Light, Inc., Newburyport, MA).
How Does nLAN Affect Circadian Rhythms in Model Systems?
Historically, the actions of light on circadian systems have been commonly categorized as either parametric or non-parametric. A non-parametric effect is exemplified by an acute resetting of circadian phase induced by a brief light pulse, and an absence of any sustained changes in any other parameters of the underlying clock oscillation. Such effects are the basis for much entrainment theory, particularly as it applies to nocturnal animals that are exposed to short intervals of bright light at dawn and dusk. Parametric actions, in contrast, are typified by ongoing modulations of clock function, such as a change in the free-running period, often caused by chronic light exposure. In reality, this conceptual distinction can be problematic, because parametric and non-parametric effects cannot always be completely distinguished. A brief light pulse, for example, can both acutely reset circadian phase and alter free-running period (51). Further, shifts in the light:dark cycle induce rapid shifts in the phase of activity but the SCN oscillatory network may be perturbed for several cycles before returning to steady state (52, 53).
Circadian rhythms in behavior are described using several common metrics, including period and amplitude. Even though different circadian assays are often used to measure one or more of these metrics, basic circadian theory suggests that these parameters are interrelated. Here we describe the effects of nLAN on some of the most common circadian measures. Throughout this review, we will use “nLAN” to refer to light levels below one lux, whether it is used to illuminate strictly during the night, as a constant condition, or as a brief pulse, and regardless of the source. For an overview of definitions of the various parameters, see these reviews: (54–56).
Effects of nLAN on Common Rhythm Parameters
Period
Period is the time it takes for the circadian oscillator to complete one cycle. The period of the internal circadian pacemaker can only be measured in conditions absent external time cues (zeitgebers) such as light or temperature cycles. Across multiple species, nLAN changes the free-running period (8, 25, 57, 58). For example, Syrian hamsters exposed to continuous complete darkness have a free running period (FRP) of just under 24 h. In contrast, in constant dim illumination (<0.01 lux, nLAN) these same animals have FRPs of ~0.3 h longer (8). These findings are consistent with one of “Aschoff's rules” (more accurately characterized as generalizations), which notes the tendency of FRPs to increase with light intensity in nocturnal rodents (59, 60).
Waveform
Each full cycle in behavioral rhythms can be divided into biological day and night, which can be assessed by monitoring the relative lengths of an organism's active (alpha, α) and rest (rho, ρ) phases. In nocturnal rodents, alpha and rho correspond to (biological) night and (biological) day, respectively, and vice versa in diurnal humans. Although less commonly assayed and reported than is free-running period, waveform (relative length of alpha and rho) is a fundamental property of a circadian rhythm and is encoded in the SCN (56, 61–65). Aschoff observed that alpha in nocturnal rodents tends to decrease with increasing light intensity. In stark contradiction of this rule, the active phase of Syrian hamsters increases by 3 h in nLAN vs. darkness (8). Effects of nLAN on alpha are discernible even under entrained conditions with varying photoperiods. In most nocturnal species examined, exposure to long winter nights – or short days – eventually yields a waveform with an elongated biological night as assessed by lengthened activity duration, melatonin secretion or SCN pattern of electrical firing and gene expression (61, 66). Upon transfer of male Siberian (Phodopus sungorus) and Syrian hamsters from summer to winter photoperiods, nLAN accelerates short-photoperiod entrainment as well as its downstream sequelae (e.g., photoperiodic regulation of reproduction) (8, 67–70).
Amplitude
Whereas the free-running rhythm of a measured rhythm unambiguously reflects the period of an underlying pacemaker, the same is not necessarily true for rhythm amplitude. The amplitude of a rhythm describes the magnitude of the change in an output signal (for example body temperature, wheel-running or SCN electrical firing) throughout one cycle. Mathematically, pacemaker amplitude has been conceptualized as related to its phase perturbability by external zeitgebers, with small amplitude pacemakers having larger amplitude phase shifting responses (71, 72). Paradoxically, short photoperiods increase the amplitude of phase shifting by bright light pulses in rodents (68, 69), suggesting a smaller pacemaker amplitude while simultaneously increasing the daily variation in SCN electrical firing (73, 74). With respect to nLAN, there is sporadic evidence of increased wheel-running amplitude in Syrian hamsters (48). In subsequent studies using slightly different running wheels, the amount of wheel-running was indistinguishable in dark vs. nLAN conditions (8). To our knowledge, wheel-running amplitude has never been observed to decrease with nLAN. Importantly from the perspective that wheel-running intensity may have feedback effects on circadian pacemakers (75), the matched activity levels in dark vs. dim nLAN excludes this feedback as the basis for observed differences in circadian period and waveform among male Syrian hamsters (8). In other contexts, nLAN-effects can be documented in the complete absence of running wheels (70).
Together, characterization of basic circadian parameters clearly demonstrates that feasibly attainable levels of darkness (e.g., <10−6 lux) and nLAN are not biologically equivalent despite the common belief that such “dim” intensities fall below the threshold for markedly altering circadian function.
nLAN as a Zeitgeber
Dim Light PRC
Brief exposure to light (i.e., light pulses) can induce phase shifts in free running organisms. Depending on the timing of these light pulses, the phase shifts can be either advancing (shifting earlier), delaying (shifting later), or of minimal magnitude. The relationship between timing of the stimulus and the resulting phase shifts is described in a phase-response curve (PRC). While the amplitude of the light PRC can vary depending on the intensity, duration, and spectral properties of light, the shape is very consistent across species (76). Typically, light induces advances at the end of the (biological) night, delays at the beginning of the biological night, and minimal phase shifts during the middle of the biological day, in both diurnal and nocturnal animals. Against a dark background, 2-h pulses of nLAN in male Syrian hamsters yielded a statistically significant PRC with an amplitude of 45 min that was generally similar in shape to that from a 15-min bright light PRC (8).
Entrainment
The ability of nLAN light pulses to induce phase shifts suggests that such illumination might be a strong enough zeitgeber to entrain mammals. The Gorman lab at UC San Diego, however, has never directly tested entrainment in a light-dark cycle alternating nLAN and complete darkness (dim-dark). Efforts by others, on the other hand, did demonstrate that mice (C57Bl/6) exposed to 12 h dim−12 h dark light cycles were able to entrain with lights as dim as 0.0005 lux, depending on the wavelength (77). Similarly, Molossid bats (Molossus molossus) were able to entrain to 0.1 lux, with half of the animals entraining to light as dim as 0.00001 lux (33). Lastly, daily 2 h pulses of 0.07 lux of “deep red” light was enough to reliably entrain female albino rats (78). In sum, even relatively short exposure to nLAN levels of light can be a zeitgeber in at least four different species.
Acute Effects of nLAN
In addition to affecting the underlying circadian pacemaker as described above, low levels of dim light can affect the output behaviors directly.
Masking + Melatonin Suppression
Masking refers to the phenomena where a stimulus directly suppresses (negative masking) or enhances (positive masking) a certain behavior below or above levels expected based on circadian phase. These changes are direct effects on clock outputs and might not necessarily change the underlying circadian pacemaker. In rodents, a common example of negative masking is the suppression of activity levels by light during the biological night. Positive masking is often seen following a cage change during the day, where activity levels are temporarily higher than typical for the time of day because of novelty-induced activity that does not reflect the underlying circadian biology (79). Common examples of masking in humans are activity-induced changes in core body temperature, light-induced increased alertness, or suppression of plasma melatonin levels by light. Even if masked responses do not necessarily originate from or affect the underlying circadian pacemaker (although in the example of light-suppressed activity in rodents or melatonin in humans, it might do both), masking is important to every circadian researcher as it might affect inferences made about the underlying pacemaker if not appropriately controlled (80, 81). Furthermore, masked behavioral or physiological signals may feed back to the core clock and alter it in subsequent cycles.
In rodents, thresholds are different for positive (increased activity by darkness) and negative (decreased activity by light) masking, and depend on wavelength (79, 82–86). In mice, the lowest intensity at which positive and negative masking was observed with 518 nm light was 109 and 1012 photon flux, respectively (77). In Siberian hamsters, nLAN was sufficient to induce strong positive and negative masking, depending on the timing and duration, although effects cannot be completely separated from entrainment effects (87).
Melatonin suppression, often considered a form of negative masking, is the most commonly measured effect of light in human research (88) and relies on the SCN (66). The threshold intensity to acutely suppress pineal melatonin in Syrian hamsters was determined to be between 0.019 and 0.186 μW/cm2, or 0.11 and 1.08 lux (89), similar to a lower threshold for 300 s 503 nm light of 3.5 × 1010 photons/cm2/s (41). In an entrainment study in Syrian hamsters, 8 h of nLAN (0.1 lux) significantly suppressed melatonin expression compared to darkness (8). Because 2 or 5 h of nLAN was not sufficient to suppress melatonin in the same animals, the effects seen with 8 h of exposure might result from differences in entrainment itself, rather than acute suppression (masking). Similarly, in young Sprague-Dawley rats maintained in light-dark cycles with various amounts of dim light at night, 0.08 μW/cm2 (0.2 lux) light suppressed plasma melatonin, while 0.06 μW/cm2 did not (90), and in female nude rats, 0.08 μW/cm2 (0.2 lux) of light at night was sufficient to attenuate nightly melatonin levels (91). Again, entrainment effects cannot be separated from acute masking. Although the relative contribution of acute and chronic exposure to (or parametric vs. non-parametric effects of) nLAN might not be definitive, it should be apparent that nLAN affects both levels of behavior and melatonin in a variety of rodent species.
Pupil Responses
Because many circadian responses are slow, difficult, invasive, and/or expensive to measure, pupillometry is commonly used as a proxy for circadian response. Light-induced pupil constriction relies on the same non-image forming visual system (e.g., photoreceptors and synaptic connections) as the circadian clock, and can therefore be a useful substitute psychophysical measure (92). Just like most measures, pupillary light responses are graded responses that depend on intensity and wavelength. In mice, reliable pupil constrictions were observed at 1010.4, 1011.4, and 1012.4 photon flux for 470, 517, and 626 nm light, respectively (77). These thresholds are roughly comparable to levels reported by others (93–95), corroborating the overall conclusion that nLAN elicits biologically relevant responses (77, 96).
nLAN as a Potentiator for Other Stimuli
The research summarized above demonstrates that very low levels of light influence both internal circadian rhythms as well as the behavioral and physiological outputs we measure to infer internal circadian conditions. Furthermore, nLAN can interact with, or potentiate, other light and non-visual stimuli. In this section, the research on indirect effects of nLAN is summarized.
Phase Angle of Entrainment
A phase angle is the relative timing between two phase markers. For the phase angle of entrainment in nocturnal animals, the timing of onset of activity is often expressed relative to lights-off transition. Non-parametric entrainment predicts that oscillators with different free-running rhythms entrain to the same zeitgeber signal with different phase angles of entrainment (97). Following the lengthening of FRP with dim light, this would predict a relatively later activity onset in a light-dim compared to a light-dark cycle. On the contrary, activity onset was observed to occur 0.8 h earlier in Siberian hamsters exposed to nLAN compared to dark controls (87).
Resetting
The phase response curve of a 15-min bright light (100 lux) pulse in male Syrian hamsters free-running in low levels of dim light does not statistically differ in shape or amplitude from the same PRC obtained in constant darkness (8). Notwithstanding this similar bright-light PRC, nLAN does facilitate re-entrainment following a phase shift (simulated jet-lag experiment) (87, 98). For example, in both Syrian and Siberian hamsters, nLAN significantly accelerated re-entrainment recovery following a 4- or 8-h phase shift compared to completely dark nights, and was observed for advances as well as delays and among animals of different ages. In the most robust case, animals exposed to dim light re-aligned activity up to 68% faster (98).
Resetting to Non-photic Cues
While light is the most potent and dominant zeitgeber for mammalian circadian rhythms, non-photic cues can also phase shift and/or entrain rodent behavior. For example, introducing a wheel-naive animal to a running wheel triggers activity. The phase shift that can be induced by this novel wheel running is amplified by nLAN (99). Similarly, a cage change can induce activity levels atypical for the circadian phase which produces a significant PRC. Amplitude of the PRC of cage-changing, however, was not significantly altered by nLAN (8).
Extreme Entrainment: T-Cycles and Bifurcation
Non-parametric entrainment theory explains entrainment by positing daily discrete phase corrections of the same magnitude as the difference between the internal and external rhythms. Real-life entrainment, however, is undoubtedly more complex than just a daily non-parametric phase resetting (100, 101). As mentioned above, the shape of the bright light PRC was not affected by an nLAN background, but re-entrainment to simulated jetlag is reliably accelerated. This observation indicates that nLAN influences re-etrainment by bright light regimes in a manner that goes beyond simple non-parametric resetting.
A near-defining feature of circadian rhythms is their narrow range of entrainment. In most mammals, entrainment to light-dark cycles much more than 2 h longer or shorter than 24 h would be considered exceptional. This range, however, can be very markedly expanded by incorporation of nLAN and/or twilight transitions. Once again, Kavanau raised the possibility of such an effect, but provided only sketchy data in support of the claim (46). More definitively, in a study of twilight transition effects in Syrian hamsters, Boulus et al. (102) demonstrated a markedly increased range of entrainment to both short and long (non-24 h) cycles (T-cycles), compared to typical instantaneous transitions between day and night conditions; however, besides ramping up or down the intensity gradually, the experimental manipulations also included a tonic exposure to nLAN throughout most of the scotophase. Without explicitly engaging the issue of this nighttime illumination, the authors attributed the enhanced range of entrainment to the twilight transitions. Having demonstrated tremendous efficacy of nLAN, we assessed whether its presence, without twilight transitions, was sufficient to extend the upper range of entrainment (we did not conduct a parallel assessment of short T-cycles). Indeed, the upper range of entrainment was significantly extended with nLAN, and some animals met entrainment criteria at cycles as long as 30 h (103). Using dim light at night, various rodent species have demonstrated reliable entrainment to cycles as extreme as 6 h on either side of 24 h (19, 103–105). This type of circadian flexibility is unprecedented in genetically intact mammals with dark nights.
As referenced above, nLAN facilitates a bifurcated pattern of entrainment in mice and two species of hamsters exposed to particular 24 h LDLD cycles, which of course is not a zeitgeber condition experienced in nature. For example, mice (C57Bl/6j) exposed to LDLD 7:5:7:5 with dark nights will generally entrain with a unimodal pattern of wheel running and treat the alternate scotophases as biological day (remain mainly inactive). In the presence of nLAN, however, most mice reorganize their behavior and divide activity between the two scotophases nearly symmetrically. Although some T24 LDLD conditions could be equivalently described as a T12 LD cycle, non-T12 LDLD conditions such as LDLD9:5:5:5 etc. also induce and maintain bifurcation. Systematic investigations of bifurcated entrainment clearly establish that it reflects temporally reorganized 24 h underlying oscillations rather than a 12 h clock (48, 106–110).
Both of these consequences of nLAN – enhanced T-cycle entrainment and behavioral rhythm bifurcation – cannot be explained by simple masking (19, 104, 106, 110–119). By the same token, neither entrainment pattern is readily understood by non-parametric entrainment theory that accurately models entrainment to more traditional experimental regimens. For example, the extended upper range of entrainment in nLAN is not explicable in terms of a proportionately increased delay portion of the light pulse PRC, nor a sufficiently large lengthening of free-running period (beyond the 0.3 h effect described above). Phase angles in dim-facilitated entrainment in non-24 h lighting conditions do not consistently follow the patterns predicted by relative length of the LD-cycle and the FRP, suggesting more complex entrainment mechanisms must play a role (19, 104, 112, 120). In addition, the acceleration of re-entrainment following simulated jetlag, which occurs for phase advances and delays of various sizes, cannot be explained by changes in free-running period without concomitant changes in the PRC amplitude.
The mechanisms by which nLAN affects circadian behavior remain to be much more deeply investigated, but nLAN is known to acutely affect electrical activity in the SCN. Specifically, electrophysiological recordings in awake Wistar rats show acute responses in SCN cellular activity to light levels as low as 0.15 lux (lowest intensity reported) (121). Additionally, some nLAN effects are mediated by the intergeniculate leaflet (122), a thalamic area with neuropeptide Y projections to the SCN and which has been implicated in integration of non-visual feedback to the SCN (75). Despite the lack of a clear mechanistic understanding, a recurring theme, beyond the scope of this review, is that nLAN may alter coupling interactions among multiple oscillators comprising the circadian pacemaker (67, 123, 124), and this change in coupling results in a more flexibly entrained oscillator to a host of conditions. Coupling remains a poorly understood dimension of circadian organization, but it is central to the functioning of a complex pacemaker. It is worth mentioning that the behavioral effects of nLAN appear often to be categorical (e.g., bifurcated or not) rather than modest extensions of entrainment parameters based on bright light entrainment theory. Thus, nLAN seems to be doing something fundamentally different than tweaking known dose-response relationships between light and phase-shifting. Collectively, these experiments demonstrate that in the right conditions, the circadian system can be much more flexible than traditional circadian theory predicts. Such flexibility is unprecedented in intact animals without genetic or pharmacological intervention or with completely dark nights.
Comparing Rodents to Humans
To properly assess how relevant the summarized research on nLAN in model organisms is for human circadian rhythms, in this section we compare the pertinent neurobiology across species. First, the mammalian circadian system is very well-conserved (76, 125), including fundamental properties of the primary oscillators. While the main body of mechanistic basic research involved nocturnal rodents (mice, rats, hamsters), circadian rhythms have been well-described in diurnal rodent species, including grass rats, degus and squirrels as well as larger mammals, including sheep and several species of non-human primates [e.g., baboon (126)]. In humans, the possibilities for mechanistic, anatomical, molecular, or neuronal studies are limited, but available data from post-mortem studies, for example, [e.g., (127–129)], confirm homology with other species. Circadian rhythms in all these species rely on a similar transcription-translation feedback loop and a functionally equivalent retinohypothalamic tract, and are orchestrated by an anatomically similar SCN with comparable cell subtypes (127, 130). Furthermore, human circadian rhythms in behavior and physiology match those of other mammalian species rather closely. Functionally, rhythms in the SCN and melatonin levels (as well as suppression and resetting by light) are similarly phased in nocturnal and diurnal species, including humans, (i.e., they are tied to environmental day/night rather than the rest/activity cycle) (131–133). Because of the similarities in circadian clock functions across species (76, 134, 135), the presumption should be that the human biological clock might, as in rodents, exhibit un(der)studied responses to low levels of light that are generally accepted to be ineffective. We will discuss the field's use of dim and dark as a control in more depth below.
Biological potency of light does not solely depend on the molecular and neurological foundations in the SCN, but also on the retina. Most mammals have three classes of photoreceptors (136). Cones are the photoreceptors used for color vision under photopic conditions (brighter light) and are the most variable between species. While primates, including humans, have three types of cones (S, M, and L, for short, medium and long wavelengths, respectively) (137), mice only have two types, lacking a long-wavelength cone found in primates and rendering mice incapable of distinguishing between green and red light (138). Albeit with less sensitivity, mice can still respond to long-wavelength light and are not blind to “red light” [reviewed in (96)]. In primates, 99% of cones are in the fovea, providing us with high-acuity vision in a small part of our visual field (139). Mice also lack a fovea, making the entire retina much more similar to the peripheral retina in primates. The majority of photoreceptors, in both primates and rodents, are the much more sensitive rods, which are primarily at play under low light conditions (140, 141). Because a primate eye is bigger than a mouse eye, the total number of photoreceptors is larger in primates. Both cones and rods have been demonstrated to contribute to circadian responses to light (142–144).
Furthermore, the mammalian retina contains a third type of photoreceptor: intrinsically photoreceptive retinal ganglion cells, or ipRGCs (143, 145–148). The much more recently-discovered ipRGCs are present in both humans and rodents, are highly conserved across species (149, 150) and are primarily responsible for the majority of non-image forming photic responses (85). In addition to their intrinsic photoreception through melanopsin, ipRGCs also receive input from other photoreceptor types (151). Combined intrinsic and extrinsic input makes ipRGCs sensitive, albeit with an increased latency, to nLAN lower than 107 photons/cm2/s, close to absolute detection limits in human vision (146, 152). This suggests that nLAN effects rely at least in part on rod-mediated photoreception, which may be routed through ipRGCs. The similarities in these two types of photoreceptors between mammalian species [review: (153, 154)] corroborate the idea that humans could be sensitive to such light levels as well.
Even if retinal circuitry for dim light reception were comparable between rodents and primates, one might question whether the inverted rest/activity cycles would render dim light at night irrelevant to diurnal animals who are sleeping with closed eyelids through much of the night. First, there is no data in rodents suggesting dim light needs to be delivered uninterrupted throughout the night. In fact, outside, under natural conditions, rodents might spend parts of their night underground, only experiencing ecological light at night during a limited window (30, 155, 156). Second, light has been delivered in sleeping humans (157–159), demonstrating that closed eyes do not fully block light or its effects on circadian biology. For example, although the purpose of the experiment was to study traditional bright light responses, brief pulses of light flashed through closed eyes while sleeping induced significant phase shifts in melatonin rhythms (159). Total transmission through adult eyelids is estimated at 0.3–3%, with more transmission for longer wavelengths (160, 161). Even if this means intensities for sleeping humans may need to be adjusted to correct for closed eyes, given the range of light levels described in nLAN studies (0.01–0.1 lux), that would still include very dim light (<3 lux), significantly below intensities of most night lights and electronic devices as well as most “dim” light used in human studies (Figure 1).
Together, comparisons of circadian neurobiology and ocular neuroanatomy provide ample evidence to believe the effects of nLAN in rodents could be relevant to humans, and that they would not be impractical or unfeasible as targets of circadian manipulation. While closed eyelids are a valid factor to consider when optimizing delivery of light, we believe the cross-species similarities are strong enough to warrant careful study of the effects of nLAN in humans.
Dim Light Studies in Humans
Most physiological effects of light, albeit not all (e.g., alpha, as discussed above), in both humans and model systems, demonstrate a characteristic intensity-dependent response, with increasing photic intensities yielding relatively greater responses. Examining the magnitude of these responses across a range of light intensities allows for the construction of a fluence response curve, with a reportable goodness of fit of the data to a sigmoid function, which provides various parameters of interest. Threshold sensitivity represents the lowest intensity of light required to elicit a detectable physiological response, and saturation occurs at the intensity at which additional light cannot increase the response further. The photic dose eliciting a half-maximum response (ED50) is also a useful point of comparison, as it occurs along the steep portion of the curve, comfortably within the range of responsiveness. Consequently, when relatively lower light levels are capable of eliciting an equivalent (ED50) response, greater photic sensitivity can be inferred.
In comparing human fluence-response curves for phase shifting and melatonin suppression to those in some of the most commonly employed model systems [but see (162) for greater taxonomic consideration], there appear to be species differences in photic sensitivity, as indicated by significantly lower values of ED50 in hamsters 0.04–1.64 melanopic lux (41, 68, 163) vs. humans ~4–60 melanopic lux (40, 42, 164–168) (see Table 2). When human studies did not optimize other elements known to exert an influence, such as spectrum and pupil dilation, relatively greater intensities of light (60–75 melanopic lux) were required to achieve a comparable half-saturation response, and this is true across different physiological effects of light (2, 40). Yet, the light levels required to elicit a response in those studies are also more likely to map on to real world applications, where white polychromatic light exposures to eyes with freely responding pupils are the norm. Most animal work with complete fluence-response curves has not optimized spectrum or pupil dilation and thus, the ~2 orders of magnitude difference in ED50s between species is likely to represent an underestimation. Nonetheless, the 560 nm narrowband nLAN stimulus typically used to illuminate the scotophase in Gorman lab experiments is orders of magnitude lower than the calculated ED50 (or even minimum threshold) for melatonin suppression in the same species. By the same logic, even after considering species differences in ED50, humans might be sensitive to light well below 1 lux. Therefore, the presented effects of nLAN underscore the idea that light in the tail of the fluence response curves could still be potent enough to elicit circadian responses, but might be overlooked by focusing on effects at ED50.
Most commonly, dim light in the nLAN (or even brighter) range of intensities appears in human studies only as a control condition and/or in contrast to a “bright light” condition [e.g., (15, 169)—see Figure 1]. But the assumption that there is no effect of nLAN on circadian physiology should be recognized as such—an assumption. First, without a no-light control group, we cannot say so definitively. Second, given the fact that ipRGCs also contribute to brightness discrimination (149), it is quite conceivable that a circadian response to a light stimulus might be affected by the contrast (fold increase) with the background, or light history, rather than absolute intensity. For example, switching between a background of 0.1 and 1 lux could conceivably be as important as the difference between a 100 and 1,000 lux stimulus. Lastly, there is emerging evidence that the precise mechanisms and pathways differ across various circadian responses (69, 77, 170–174). This also applies to the shape of the fluence response curve—for example, while effects of light may be largely linear for phase-shifting or melatonin suppression, the effects of nLAN, which appear to be largely parametric, are likely non-linear. The field of circadian research is littered with designs based on these assumptions, whether implicit or explicit.
In classic studies of the physiological effects of light in humans, participants are often maintained under dim light conditions or darkness prior to administration of a light pulse in order to control for the potential effects of photic history. Though the intensity and duration of that period of adaptation varies across studies, research that has systematically examined the effects of photic history in humans has shown robust effects. Most of these studies have altered daytime photic exposure and then, examined subsequent response to a test pulse during the biological night. For example, Chang et al. (169, 175) had participants spend 3 days in the laboratory under either dim light (1 lux) or relatively brighter light (90 lux) during waking hours, while scotopic levels were kept very dim (<0.1 lux) across both conditions. When participants were subsequently exposed to a 6.5 h light pulse (90 lux) ~1 h prior to habitual bedtime, a 40 min greater circadian phase delay and increased alertness occurred with pre-exposure to daytime dim vs. brighter light. Earlier studies similarly had participants exposed to more or less daytime light in the week preceding a bright light test pulse during the biological night, and there was significantly increased melatonin suppression and phase shifting after the dimmer daytime condition (176–178). In contrast, within a single, much shorter time frame (2 h), early in the scotophase and during the biological night, dim light served to attenuate the melatonin suppression response to a subsequent 90 min test pulse as compared to a completely dark adaptation period (179). Together, these findings are consistent with the recommendation of a high day-night contrast, in terms of biological potency, in order to clearly signal time of day to the circadian timing system and maximize physiological responses to light [e.g., (14, 180)]; however, they leave open the question as to whether complete darkness or nLAN is an optimal scotophase condition.
Two unique examples of studies that included human exposure to nLAN include work from Wright et al. (181, 182), in which participants were exposed to only natural sources of light (including moon and starlight) while tent camping. In the first paper, circadian outputs for this week were compared, within participants, to a more typical week that included work, school, self-selected sleep schedules, and time spent in built environments with electrical lighting. The smaller phase angle of entrainment to the solar day during the camping period of only natural light exposure was primarily attributed to less electrical light at night and more light (from the sun) during the day, yet nLAN cannot be discounted as a potential contributing factor. Indeed, in the second study, nocturnal light while camping is characterized in more detail, and they report sensor-derived night light levels in the 500–600 nm range between 0.1 and 1 lux (in addition to intermittent light from a campfire) (182).
To our knowledge, there has never been a direct examination of the effects of very dim vs. complete darkness throughout the scotophase in human studies of the physiological effects of light. Considering the varied background conditions in the seminal human work on the topic, it may be possible to retrospectively compare a variety of “dark” scotophase conditions across experiments in order to glean whether or not there are potential effects of nLAN in humans that are similar to what has been established in model systems.
Summary and Recommendations to the Field
While it remains to be rigorously examined, there is good reason to suspect that the potent effects of very dim light (akin to moon and starlight intensities) summarized here have translational potential for human circadian research, including the fact that the tremendous overlap in circadian physiology and responses between species make it likely that such effects are possible in humans. However, even if these effects do not directly translate (i.e., if it is established later that these lower levels of light do not markedly affect the human circadian system in these ways, or indeed, in any discernible way), they nevertheless provide a window into the potential for a latent plasticity that may exist in humans and may be inducible via other mechanisms, which have yet to be determined (119). Keeping both of these notions in mind, but focusing on the former, the following section includes some recommendations for future work for circadian researchers focused on mammalian systems.
Measurement and Reporting of Light
This review focuses on photic intensity; however, light can vary along several other important dimensions, including spectral properties, duration, and directionality. All of these parameters have been shown to affect biological potency. Recent progress has been made, via an internationally balloted consensus-based process, in developing a standardized method of measuring and reporting light for non-image forming physiological effects of light [CIE S 026:2018 (14)]. Essentially, the intensity for each of the five α -opic photoreceptors (S-cone-opic, M-cone-opic, L-cone-opic, Rhodopic; Melanopic) is determined, allowing for the assessment of the relative contribution to a given response; this is conceptually similar to and builds upon the introduction of melanopic lux (13). Under typical lighting conditions, melanopic contribution is the dominant photoreceptor mediating photic input for the physiological effects of light in humans, such as melatonin suppression and phase shifting (183–185). Thus, when quantifying photic stimuli for these physiological effects of light, stimuli should be characterized in terms of melanopic Equivalent Daylight Illuminance (EDI), which is also reported in units of lux (14). While we support these new metrics for quantification of light for non-visual responses, converting previously reported photopic lux values into precise melanopic lux/EDI is often not feasible due to insufficient information about the spectral quality of the light stimulus. The Lucas and CIE toolboxes (13, 14), however, support such conversions by providing standardized or estimated SPD for several light sources. (Figure 1; see below for recommendations for reporting light in research papers). Furthermore, the primary purpose of this review is to emphasize the potency of nLAN, which has largely been assumed to be subthreshold for affecting the mammalian circadian system, regardless of spectrum. The research summarized above should make it abundantly clear that light levels multiple orders of magnitude below 1 lux are sufficient to alter circadian rhythms both directly and indirectly.
In all work going forward, researchers should take pains to ensure that lighting conditions are reproducible and convertible. This requires detailed reporting of the set up, the distance and gaze of the organism, placement of sensors, the measurement instruments used (including their ranges of accuracy, the exact sensor-heads used, the ability to set a 0-reference point, etc.), and any other factor that could influence the effect of light (e.g., directionality, dilated pupils, etc.), see (186). Many labs, however, are likely ill-equipped to accurately measure light levels in the nLAN range. At a minimum, researchers should state that light levels were below levels of detection by their equipment, state what those levels are, and report details on any effort taken to reduce light levels, such as removing or blocking light sources. The availability of open access and supplemental options mean a much more detailed account of lighting set ups need not be subject to space or word limit concerns. At a minimum, the specifications of the light source need to be described. For example, light sources that can be described as “white light” or “white fluorescent” can span at least an order of magnitude in their biological potency (183, 187). Further, as discussed above, light levels should be reported in units that can be later converted (e.g., spectral power distributions of all light sources) if reporting metrics change, as is likely as more is learned (14). For example, the bulk of the nLAN work described here was conducted before the emergence of melanopic lux (13, 14). However, because the spectral composition and irradiance are reported in our work, we can now calculate the melanopic lux, or any other metric that emerges from the field subsequently (see Table 1 and Figure 1).
Standardization of Terminology
Standardization of language both within the circadian and lighting communities surrounding light levels, especially lower levels, is crucial, both in our science communications as well as public health outreach and messaging. In the future, it might be useful to develop a set of agreed upon terms to further narrow the range of intensities currently termed as “dim light.” For example, “dim” has been used variously to describe values between 0.0001 and 500 lux (see Figure 1), which spans many orders of magnitude. In this paper, we have used the term “nLAN” throughout to describe intensities of light no brighter than naturally occurring nighttime light levels.
As a field, our current recommendation that “nights should be completely dark,” may warrant further consideration. Importantly, solar night is not naturally completely dark, and while the total absence of light is perhaps a more simple aim than prescribing a sweet-spot range of dim photic intensities that do more good than harm, absolutely no light at night may not always be feasible, optimal, or desirable. Furthermore, the lighting industry may play an important role in helping to optimize not just our days but our nights as well, with the development of novel technologies that capitalize on these more nuanced understandings.
Reconsidering Old Data and Designing New Studies
Existing data sets and conclusions drawn from them may be reconsidered in light of the evidence presented here. It may be possible to conduct a systematic review and/or meta-analysis of data from near-scotopic conditions, either within or across species.
Additionally, while painstaking, new studies of the biological effects of light in humans need to include sufficient data points to measure responses at different intensities, including no detectable light, and time courses.
Recent discoveries show that not all physiological effects of light rely on identical mechanisms [e.g., alertness vs. melatonin suppression (41, 42, 69, 77, 85, 95, 170–174)]. Thus, ED50s of one circadian response should not be assumed to generalize to a different response. The same caution applies to sensitivity values reflected in the tails of fluence response curves, where estimation is less reliable. To better understand these response differences, further studies aiming to better understand relative photoreceptor contribution and to identify practically important dose response parameters should ideally include measurements across multiple responses (e.g., pupillary constriction, entrainment) in the same subject under the same experimental conditions. Where collection of multiple endpoints might not be feasible, the use of standardized, and replicable, methodologies becomes even more critical to enable between study comparisons.
Finally, should investigators need dim illumination for practical purposes, given the relative biological potency of short wavelengths, including at relatively “dim” levels (Figure 1), we recommend the use of the dimmest light, most-depleted of short wavelengths, possible. For example, 10 lux with a 650 nm LED, more than is needed for comfortable vision, is only 0.01 melanopic lux, as opposed to 10 lux fluorescent light (~3,000 K), which is 6.2 melanopic lux (Figure 1). While the former light source is undoubtedly less potent, researchers should nevertheless bear in mind that, in at least one case, “deep red” light levels as low as 0.07 lux were sufficient to elicit behavioral entrainment in albino rats (78). Furthermore, this same lighting stimulus was described by the authors as insufficient to aid researchers with animal handling. Thus, as with many experimental design considerations, there are trade-offs between rigor and practicality; however, there may be no such thing as “dim enough” when trying to minimize effects of background light – any amount of light may potentially affect the circadian system. Therefore, investigators should both consider and report light levels in every condition, including those that may have otherwise been simply described as “dim” or “dark.”
Author Contributions
GG, TW, EH, and MG: conceptualization. TW, EH, and GG: analysis. TW, EH, GG, and MG: writing. GG: funding acquisition. All authors contributed to the article and approved the submitted version.
Funding
Conversions of light levels and associated analyses were supported by the Department of Energy #DE-EE0008206 (GG). Analyses and writing associated with background, review and translational concepts, as well as open access publication fees, were supported by the Office of Naval Research #N6290919MP00030 (GG) and by NIH/NINDS #R01NS102962 (TW).
Disclaimer
The opinions and assertions expressed herein are those of the authors and do not reflect the official policy or position of the Uniformed Services University or the Department of Defense.
Conflict of Interest
The authors declare that the research was conducted in the absence of any commercial or financial relationships that could be construed as a potential conflict of interest.
Acknowledgments
The authors would like to acknowledge Sara Bessman and Michael Herf for their continued support with photic conversions to alpha-opic illuminance values, particularly for the much lower intensities that required modification to Toolbox settings.
References
1. Duffy JF, Czeisler CA. Effect of light on human circadian physiology. Sleep Med Clin. (2009) 4:165–77. doi: 10.1016/j.jsmc.2009.01.004
2. Cajochen C. Alerting effects of light. Sleep Med Rev. (2007) 11:453–64. doi: 10.1016/j.smrv.2007.07.009
3. Brainard GC, Sliney D, Hanifin JP, Glickman G, Byrne B, Greeson JM, et al. Sensitivity of the human circadian system to short-wavelength (420-nm) light. J Biol Rhythms. (2008) 23:379–86. doi: 10.1177/0748730408323089
4. Münch M, Wirz-Justice A, Brown SA, Kantermann T, Martiny K, Stefani O, et al. The role of daylight for humans: gaps in current knowledge. Clocks Sleep. (2020) 2:61–85. doi: 10.3390/clockssleep2010008
5. Plano SA, Casiraghi LP, García Moro P, Paladino N, Golombek DA, Chiesa JJ. Circadian and metabolic effects of light: implications in weight homeostasis and health. Front Neurol. (2017) 8:558. doi: 10.3389/fneur.2017.00558
6. González MMC. Dim light at night and constant darkness: two frequently used lighting conditions that jeopardize the health and well-being of laboratory rodents. Front Neurol. (2018) 9:609. doi: 10.3389/fneur.2018.00609
7. Evans JA, Elliott JA, Gorman MR. Individual differences in circadian waveform of Siberian hamsters under multiple lighting conditions. J Biol Rhythms. (2012) 27:410–9. doi: 10.1177/0748730412455915
8. Evans JA, Elliott JA, Gorman MR. Circadian effects of light no brighter than moonlight. J Biol Rhythms. (2007) 22:356–67. doi: 10.1177/0748730407301988
9. Cajochen C, Altanay-Ekici S, Münch M, Frey S, Knoblauch V, Wirz-Justice A. Evidence that the lunar cycle influences human sleep. Curr Biol. (2013) 23:1485–8. doi: 10.1016/j.cub.2013.06.029
10. Della Monica C, Atzori G, Dijk D-J. Effects of lunar phase on sleep in men and women in Surrey. J Sleep Res. (2015) 24:687–94. doi: 10.1111/jsr.12312
11. Casiraghi L, Spiousas I, Dunster G, McGlothlen K, Fernández-Duque E, Valeggia C, et al. Moonstruck sleep: synchronization of human sleep with the moon cycle under natural conditions. bioRxiv. (2020) doi: 10.1101/2020.06.01.128728
12. de la Iglesia HO, Fernández-Duque E, Golombek DA, Lanza N, Duffy JF, Czeisler CA, et al. Access to electric light is associated with shorter sleep duration in a traditionally hunter-gatherer community. J Biol Rhythms. (2015) 30:342–50. doi: 10.1177/0748730415590702
13. Lucas RJ, Peirson SN, Berson DM, Brown TM, Cooper HM, Czeisler CA, et al. Measuring and using light in the melanopsin age. Trends Neurosci. (2014) 37:1–9. doi: 10.1016/j.tins.2013.10.004
14. CIE S 026:2018: Cie System For Metrology Of Optical Radiation For Iprgc-influenced Responses To Light. Vienna (2018). Available online at: http://www.cie.co.at/publications/cie-system-metrology-optical-radiation-iprgc-influenced-responses-light-0
15. St Hilaire MA, Gooley JJ, Khalsa SBS, Kronauer RE, Czeisler CA, Lockley SW. Human phase response curve to a 1 h pulse of bright white light. J Physiol. (2012) 590:3035–45. doi: 10.1113/jphysiol.2012.227892
16. Wright KP, Hughes RJ, Kronauer RE, Dijk DJ, Czeisler CA. Intrinsic near-24-h pacemaker period determines limits of circadian entrainment to a weak synchronizer in humans. Proc Natl Acad Sci USA. (2001) 98:14027–32. doi: 10.1073/pnas.201530198
17. Phillips AJK, Vidafar P, Burns AC, McGlashan EM, Anderson C, Rajaratnam SMW, et al. High sensitivity and interindividual variability in the response of the human circadian system to evening light. Proc Natl Acad Sci. (2019) 116:201901824. doi: 10.1073/pnas.1901824116
18. Evans JA, Elliott JA, Gorman MR. Dynamic interactions between coupled oscillators within the hamster circadian pacemaker. Behav Neurosci. (2010) 124:87–96. doi: 10.1037/a0018088
19. Walbeek TJ, Gorman MR. Simple lighting manipulations facilitate behavioral entrainment of mice to 18-h days. J Biol Rhythms. (2017) 32:309–22. doi: 10.1177/0748730417718347
20. Duffy JF, Dijk D-J. Getting through to circadian oscillators: why use constant routines? J Biol Rhythms. (2002) 17:4–13. doi: 10.1177/074873002129002294
21. Bedrosian TA, Fonken LK, Walton JC, Nelson RJ. Chronic exposure to dim light at night suppresses immune responses in Siberian hamsters. Biol Lett. (2011) 7:468–71. doi: 10.1098/rsbl.2010.1108
22. Stenvers DJ, van Dorp R, Foppen E, Mendoza J, Opperhuizen A-L, Fliers E, et al. Dim light at night disturbs the daily sleep-wake cycle in the rat. Sci Rep. (2016) 6:35662. doi: 10.1038/srep35662
23. Opperhuizen A-L, van Kerkhof LWM, Proper KI, Rodenburg W, Kalsbeek A. Rodent models to study the metabolic effects of shiftwork in humans. Front Pharmacol. (2015) 6:50. doi: 10.3389/fphar.2015.00050
24. Smolensky MH, Sackett-Lundeen LL, Portaluppi F. Nocturnal light pollution and underexposure to daytime sunlight: complementary mechanisms of circadian disruption and related diseases. Chronobiol Int. (2015) 32:1029–48. doi: 10.3109/07420528.2015.1072002
25. Aschoff J. Circadian rhythms: influences of internal and external factors on the period measured in constant conditions. Z Tierpsychol. (1979) 49:225–49. doi: 10.1111/j.1439-0310.1979.tb00290.x
26. Scherbarth F, Steinlechner S. The annual activity pattern of djungarian hamsters (Phodopus sungorus) is affected by wheel-running activity. Chronobiol Int. (2008) 25:905–22. doi: 10.1080/07420520802544514
27. Wolfe JL, Tan Summerlin C. The influence of lunar light on nocturnal activity of the old-field mouse. Anim Behav. (1989) 37:410–4. doi: 10.1016/0003-3472(89)90088-2
28. Daly M, Behrends PR, Wilson MI, Jacobs LF. Behavioural modulation of predation risk: moonlight avoidance and crepuscular compensation in a nocturnal desert rodent, Dipodomys merriami. Anim Behav. (1992) 44:1–9. doi: 10.1016/S0003-3472(05)80748-1
29. Fernández-Duque E, de la Iglesia H, Erkert HG. Moonstruck primates: owl monkeys (Aotus) need moonlight for nocturnal activity in their natural environment. PLoS ONE. (2010) 5:e12572. doi: 10.1371/journal.pone.0012572
30. Smarr BL, Schwartz MD, Wotus C, de la Iglesia HO. Re-examining “Temporal Niche.” Integr Comp Biol. (2013) 53:165–74. doi: 10.1093/icb/ict055
31. Hoffmann J, Palme R, Eccard JA. Long-term dim light during nighttime changes activity patterns and space use in experimental small mammal populations. Environ Pollut. (2018) 238:844–51. doi: 10.1016/j.envpol.2018.03.107
32. Erkert HG. Der Einfluß des Mondlichtes auf die Aktivitätsperiodik nachtaktiver Säugetiere. Oecologia. (1974) 14:269–87. doi: 10.1007/BF01039797
33. Erkert HG. Extremely low threshold for photic entrainment of circadian activity rhythms in molossid bats (Molossus molossus; Chiroptera—Molossidae). Mamm Biol. (2004) 69:361–74. doi: 10.1078/1616-5047-00158
34. Erkert HG, Bay FA, Kracht S. Zeitgeber induced modulation of activity patterns in nocturnal mammals (Chiroptera). Experientia. (1976) 32:560–2. doi: 10.1007/BF01990160
35. Erkert HG, Gröber J. Direct modulation of activity and body temperature of owl monkeys (Aotus lemurinus griseimembra) by low light intensities. Folia Primatol. (1986) 47:171–88. doi: 10.1159/000156276
36. Lin MC, Kripke DF, Perry BL, Berga SL. Night light alters menstrual cycles. Psychiatry Res. (1990) 33:135–8. doi: 10.1016/0165-1781(90)90067-F
37. Kronfeld-Schor N, Dominoni D, de la Iglesia H, Levy O, Herzog ED, Dayan T, et al. Chronobiology by moonlight. Proc R Soc B Biol Sci. (2013) 280:20123088. doi: 10.1098/rspb.2012.3088
38. Kyba CCM, Mohar A, Posch T. How bright is moonlight? Astron Geophys. (2017) 58:1.31–2. doi: 10.1093/astrogeo/atx025
39. Hofstetter JR, Hofstetter AR, Hughes AM, Mayeda AR. Intermittent long-wavelength red light increases the period of daily locomotor activity in mice. J Circadian Rhythms. (2005) 3:8. doi: 10.1186/1740-3391-3-8
40. Zeitzer JM, Dijk DJ, Kronauer RE, Brown EN, Czeisler CA. Sensitivity of the human circadian pacemaker to nocturnal light: melatonin phase resetting and suppression. J Physiol. (2000) 526:695–702. doi: 10.1111/j.1469-7793.2000.00695.x
41. Nelson DE, Takahashi JS. Comparison of visual sensitivity for suppression of pineal melatonin and circadian phase-shifting in the golden hamster. Brain Res. (1991) 554:272–7. doi: 10.1016/0006-8993(91)90200-F
42. Gooley JJ, Rajaratnam SMW, Brainard GC, Kronauer RE, Czeisler CA, Lockley SW. Spectral responses of the human circadian system depend on the irradiance and duration of exposure to light. Sci Transl Med. (2010) 2:31ra33. doi: 10.1126/scitranslmed.3000741
43. Walmsley L, Hanna L, Mouland J, Martial F, West A, Smedley AR, et al. Colour as a signal for entraining the mammalian circadian clock. PLoS Biol. (2015) 13:e1002127. doi: 10.1371/journal.pbio.1002127
44. Kavanau JL. Activity and orientational responses of white-footed mice to light. Nature. (1968) 218:245–52. doi: 10.1038/218245a0
45. Kavanau JL. Behavior of captive white-footed mice. Science. (1967) 155:1623–39. doi: 10.1126/science.155.3770.1623
46. Kavanau JL. Activity patterns on regimes employing artificial twilight transitions. Experientia. (1962) 18:382–4. doi: 10.1007/BF02172265
47. Rich C, Longcore T. Ecological Consequences of Artificial Night Lighting. Washington, DC: Island Press (2006).
48. Gorman MR, Elliott JA, Evans JA. Plasticity of hamster circadian entrainment patterns depends on light intensity. Chronobiol Int. (2003) 20:233–48. doi: 10.1081/CBI-120018576
49. Erkert HG, Kracht S. Evidence for ecological adaptation of circadian systems. Oecologia. (1978) 32:71–8. doi: 10.1007/BF00344690
50. Sanders D, Frago E, Kehoe R, Patterson C, Gaston KJ. A meta-analysis of biological impacts of artificial light at night. Nat Ecol Evol. (2020) 5:74–81. doi: 10.1038/s41559-020-01322-x
51. Comas M, Beersma DGM, Spoelstra K, Daan S. Phase and period responses of the circadian system of mice (Mus musculus) to light stimuli of different duration. J Biol Rhythms. (2006) 21:362–72. doi: 10.1177/0748730406292446
52. Nicholls SK, Casiraghi LP, Wang W, Weber ET, Harrington ME. Evidence for internal desynchrony caused by circadian clock resetting. Yale J Biol Med. (2019) 92:259–70.
53. Reddy AB, Field MD, Maywood ES, Hastings MH. Differential resynchronisation of circadian clock gene expression within the suprachiasmatic nuclei of mice subjected to experimental jet lag. J Neurosci. (2002) 22:7326–30. doi: 10.1523/JNEUROSCI.22-17-07326.2002
54. Kao K, Loi J, Sanderson H, Kim J, Lee J, Nudell V, et al. An Introduction to Chronobiology. The BioClock Studio (2016). Available online at: https://ccb.ucsd.edu/the-bioclock-studio/education-resources/basics/index.html (accessed November 2, 2020).
55. Wirz-Justice A. How to measure circadian rhythms in humans. Medicographia. (2007) 29:84–90. doi: 10.1111/j.1479-8425.2006.00195.x
56. Gorman MR, Harrison EM, Evans JA. Circadian waveform and its significance for clock organization and plasticity. In: Kumar V, editor. Biological Timekeeping: Clocks, Rhythms and Behaviour. New Delhi: Springer India (2017). p. 59–79. doi: 10.1007/978-81-322-3688-7_4
57. Butler MP, Karatsoreos IN, LeSauter J, Silver R. Dose-dependent effects of androgens on the circadian timing system and its response to light. Endocrinology. (2012) 153:2344–52. doi: 10.1210/en.2011-1842
58. Boulos Z. Wavelength dependence of light-induced phase shifts and period changes in hamsters. Physiol Behav. (1995) 57:1025–33. doi: 10.1016/0031-9384(95)00015-B
59. Aschoff J. Exogenous and endogenous components in circadian rhythms. Cold Spring Harb Symp Quant Biol. (1960) 25:11–28. doi: 10.1101/SQB.1960.025.01.004
60. Pittendrigh CS. Circadian rhythms and the circadian organization of living systems. Cold Spring Harb Symp Quant Biol. (1960) 25:159–84. doi: 10.1101/SQB.1960.025.01.015
61. Coomans CP, Ramkisoensing A, Meijer JH. The suprachiasmatic nuclei as a seasonal clock. Front Neuroendocrinol. (2015) 37:29–42. doi: 10.1016/j.yfrne.2014.11.002
62. Sumova A, Travnickova Z, Peters R, Schwartz WJ, Illnerova H. The rat suprachiasmatic nucleus is a clock for all seasons. Proc Natl Acad Sci. (1995) 92:7754–8. doi: 10.1073/pnas.92.17.7754
63. Schwartz WJ, de la Iglesia HO, Zlomanczuk P, Illnerová H. Encoding le quattro stagioni within the mammalian brain: photoperiodic orchestration through the suprachiasmatic nucleus. J Biol Rhythms. (2001) 16:302–11. doi: 10.1177/074873001129002024
64. Wehr TA, Moul DE, Barbato G, Giesen HA, Seidel JA, Barker C, et al. Conservation of photoperiod-responsive mechanisms in humans. Am J Physiol Integr Comp Physiol. (1993) 265:R846–57. doi: 10.1152/ajpregu.1993.265.4.R846
65. Illnerová H, Hoffmann K, Vanecek J. Adjustment of pineal melatonin and N-acetyltransferase rhythms to change from long to short photoperiod in the Djungarian hamster Phodopus sungorus. Neuroendocrinology. (1984) 38:226–31. doi: 10.1159/000123895
66. Arendt J. Managing jet lag: some of the problems and possible new solutions. Sleep Med Rev. (2009) 13:249–56. doi: 10.1016/j.smrv.2008.07.011
67. Evans JA, Elliott JA, Gorman MR. Photoperiod differentially modulates photic and nonphotic phase response curves of hamsters. Am J Physiol Regul Integr Comp Physiol. (2004) 286:R539–46. doi: 10.1152/ajpregu.00456.2003
68. Glickman GL, Webb IC, Elliott JA, Baltazar RM, Reale ME, Lehman MN, et al. Photic sensitivity for circadian response to light varies with photoperiod. J Biol Rhythms. (2012) 27:308–18. doi: 10.1177/0748730412450826
69. Glickman GL, Harrison EM, Elliott JA, Gorman MR. Increased photic sensitivity for phase resetting but not melatonin suppression in Siberian hamsters under short photoperiods. Horm Behav. (2014) 65:301–7. doi: 10.1016/j.yhbeh.2014.01.002
70. Gorman MR, Elliott JA. Dim nocturnal illumination alters coupling of circadian pacemakers in Siberian hamsters, Phodopus sungorus. J Comp Physiol A Neuroethol Sens Neural Behav Physiol. (2004) 190:631–9. doi: 10.1007/s00359-004-0522-7
71. Abraham U, Granada AE, Westermark PO, Heine M, Kramer A, Herzel H. Coupling governs entrainment range of circadian clocks. Mol Syst Biol. (2010) 6:438. doi: 10.1038/msb.2010.92
72. Schmal C, Myung J, Herzel H, Bordyugov G. A theoretical study on seasonality. Front Neurol. (2015) 6:94. doi: 10.3389/fneur.2015.00159
73. vanderLeest HT, Rohling JHT, Michel S, Meijer JH. Phase shifting capacity of the circadian pacemaker determined by the SCN neuronal network organization. PLoS ONE. (2009) 4:e4976. doi: 10.1371/journal.pone.0004976
74. VanderLeest HT, Houben T, Michel S, Deboer T, Albus H, Vansteensel MJ, et al. Seasonal encoding by the circadian pacemaker of the SCN. Curr Biol. (2007) 17:468–73. doi: 10.1016/j.cub.2007.01.048
75. Janik D, Mikkelsen JD, Mrosovsky N. Cellular colocalization of Fos and neuropeptide Y in the intergeniculate leaflet after nonphotic phase-shifting events. Brain Res. (1995) 698:137–45. doi: 10.1016/0006-8993(95)00878-T
76. Kronauer RE, Hilaire MA, Rahman SA, Czeisler CA, Klerman EB. An exploration of the temporal dynamics of circadian resetting responses to short- and long-duration light exposures: cross-species consistencies and differences. J Biol Rhythms. (2019) 34:497–514. doi: 10.1177/0748730419862702
77. Butler MP, Silver R. Divergent photic thresholds in the non-image-forming visual system: entrainment, masking and pupillary light reflex. Proc Biol Sci. (2011) 278:745–50. doi: 10.1098/rspb.2010.1509
78. McCormack CE, Sontag CR. Entrainment by red light of running activity and ovulation rhythms of rats. Am J Physiol. (1980) 239:R450–3. doi: 10.1152/ajpregu.1980.239.5.R450
79. Mrosovsky N. In praise of masking: behavioural responses of retinally degenerate mice to dim light. Chronobiol Int. (1994) 11:343–8. doi: 10.3109/07420529409057251
80. Marques MD, Waterhouse JM. Masking and the evolution of circadian rhythmicity. Chronobiol Int. (1994) 11:146–55. doi: 10.3109/07420529409057234
81. Waterhouse J, Minors D, Akerstedt T, Hume K, Kerkhof G. Circadian rhythm adjustment: difficulties in assessment caused by masking. Pathol Biol (Paris). (1996) 44:205–7.
82. Edelstein K, Mrosovsky N. Behavioral responses to light in mice with dorsal lateral geniculate lesions. Brain Res. (2001) 918:107–12. doi: 10.1016/S0006-8993(01)02966-3
83. Redlin U, Mrosovsky N. Masking of locomotor activity in hamsters. J Comp Physiol A. (1999) 184:429–37. doi: 10.1007/s003590050342
84. Redlin U, Vrang N, Mrosovsky N. Enhanced masking response to light in hamsters with IGL lesions. J Comp Physiol A. (1999) 184:449–56. doi: 10.1007/s003590050344
85. Hattar S, Lucas RJ, Mrosovsky N, Thompson S, Douglas RH, Hankins MW, et al. Melanopsin and rod-cone photoreceptive systems account for all major accessory visual functions in mice. Nature. (2003) 424:76–81. doi: 10.1038/nature01761
86. Thompson S, Foster RG, Stone EM, Sheffield VC, Mrosovsky N. Classical and melanopsin photoreception in irradiance detection: negative masking of locomotor activity by light. Eur J Neurosci. (2008) 27:1973–9. doi: 10.1111/j.1460-9568.2008.06168.x
87. Frank DW, Evans JA, Gorman MR. Time-dependent effects of dim light at night on re-entrainment and masking of hamster activity rhythms. J Biol Rhythms. (2010) 25:103–12. doi: 10.1177/0748730409360890
88. Tähkämö L, Partonen T, Pesonen A-K. Systematic review of light exposure impact on human circadian rhythm. Chronobiol Int. (2019) 36:151–70. doi: 10.1080/07420528.2018.1527773
89. Brainard GC, Richardson BA, Petterborg LJ, Reiter RJ. The effect of different light intensities on pineal melatonin content. Brain Res. (1982) 233:75–81. doi: 10.1016/0006-8993(82)90931-3
90. Dauchy RT, Dauchy EM, Tirrell RP, Hill CR, Davidson LK, Greene MW, et al. Dark-phase light contamination disrupts circadian rhythms in plasma measures of endocrine physiology and metabolism in rats. Comp Med. (2010) 60:348–56.
91. Blask DE, Dauchy RT, Dauchy EM, Mao L, Hill SM, Greene MW, et al. Light exposure at night disrupts host/cancer circadian regulatory dynamics: impact on the warburg effect, lipid signaling and tumor growth prevention. PLoS ONE. (2014) 9:e102776. doi: 10.1371/journal.pone.0102776
92. Münch M, Léon L, Collomb S, Kawasaki A. Comparison of acute non-visual bright light responses in patients with optic nerve disease, glaucoma and healthy controls. Sci Rep. (2015) 5:15185. doi: 10.1038/srep15185
93. Jain V, Srivastava I, Palchaudhuri S, Goel M, Sinha-Mahapatra SK, Dhingra NK. Classical photoreceptors are primarily responsible for the pupillary light reflex in mouse. PLoS ONE. (2016) 11:e0157226. doi: 10.1371/journal.pone.0157226
94. Keenan WT, Rupp AC, Ross RA, Somasundaram P, Hiriyanna S, Wu Z, et al. A visual circuit uses complementary mechanisms to support transient and sustained pupil constriction. Elife. (2016) 5:15392. doi: 10.7554/eLife.15392
95. Lucas RJ, Douglas RH, Foster RG. Characterization of an ocular photopigment capable of driving pupillary constriction in mice. Nat Neurosci. (2001) 4:621–6. doi: 10.1038/88443
96. Peirson SN, Brown LA, Pothecary CA, Benson LA, Fisk AS. Light and the laboratory mouse. J Neurosci Methods. (2018) 300:26–36. doi: 10.1016/j.jneumeth.2017.04.007
97. Granada AE, Bordyugov G, Kramer A, Herzel H. Human chronotypes from a theoretical perspective. PLoS ONE. (2013) 8:e59464. doi: 10.1371/journal.pone.0059464
98. Evans JA, Elliott JA, Gorman MR. Dim nighttime illumination accelerates adjustment to timezone travel in an animal model. Curr Biol. (2009) 19:R156–7. doi: 10.1016/j.cub.2009.01.023
99. Evans JA, Elliott JA, Gorman MR. Circadian entrainment and phase resetting differ markedly under dimly illuminated versus completely dark nights. Behav Brain Res. (2005) 162:116–26. doi: 10.1016/j.bbr.2005.03.014
100. Pohl H. Characteristics and variability in entrainment of circadian rhythms to light in diurnal rodents. In: Aschoff J, Daan S, Groos GA, editors. Vertebrate Circadian Systems. Proceedings in Life Sciences. Berlin; Heidelberg: Springer (1982). doi: 10.1007/978-3-642-68651-1_36
101. Pittendrigh CS, Daan S. A functional analysis of circadian pacemakers in nocturnal rodents—IV. Entrainment: pacemaker as clock. J Comp Physiol A. (1976) 106:291–331. doi: 10.1007/BF01417859
102. Boulos Z, Macchi MM, Terman M. Twilights widen the range of photic entrainment in hamsters. J Biol Rhythms. (2002) 17:353–63. doi: 10.1177/074873002129002654
103. Gorman MR, Kendall M, Elliott JA. Scotopic illumination enhances entrainment of circadian rhythms to lengthening light:dark cycles. J Biol Rhythms. (2005) 20:38–48. doi: 10.1177/0748730404271573
104. Harrison EM, Walbeek TJ, Sun J, Johnson J, Poonawala Q, Gorman MR. Extraordinary behavioral entrainment following circadian rhythm bifurcation in mice. Sci Rep. (2016) 6:38479. doi: 10.1038/srep38479
105. Chiesa JJ, Anglès-Pujolràs M, Díez-Noguera A, Cambras T. Activity rhythm of golden hamster (Mesocricetus auratus) can be entrained to a 19-h light-dark cycle. Am J Physiol Regul Integr Comp Physiol. (2005) 289:R998–1005. doi: 10.1152/ajpregu.00139.2005
106. Gorman MR, Elliott JA. Entrainment of 2 subjective nights by daily light:dark:light:dark cycles in 3 rodent species. J Biol Rhythms. (2003) 18:502–12. doi: 10.1177/0748730403260219
107. Gorman MR, Steele NA. Phase angle difference alters coupling relations of functionally distinct circadian oscillators revealed by rhythm splitting. J Biol Rhythms. (2006) 21:195–205. doi: 10.1177/0748730406287665
108. Gorman MR, Evans JA, Elliott JA. Potent circadian effects of dim illumination at night in hamsters. Chronobiol Int. (2006) 23:245–50. doi: 10.1080/07420520500521905
109. Walbeek TJ, Joye DAM, Mishra I, Gorman MR. Physiological, behavioral and environmental factors influence bifurcated circadian entrainment in mice. Physiol Behav. (2019) 210:112625. doi: 10.1016/j.physbeh.2019.112625
110. Raiewski EE, Elliott JA, Evans JA, Glickman GL, Gorman MR. Twice daily melatonin peaks in Siberian but not Syrian hamsters under 24 h light:dark:light:dark cycles. Chronobiol Int. (2012) 29:1206–15. doi: 10.3109/07420528.2012.719965
111. Sun J, Joye DAM, Farkas AH, Gorman MR. Photoperiodic requirements for induction and maintenance of rhythm bifurcation and extraordinary entrainment in male mice. Clocks Sleep. (2019) 1:290–305. doi: 10.3390/clockssleep1030025
112. Walbeek TJ, Harrison EM, Soler RR, Gorman MR. Enhanced circadian entrainment in mice and its utility under human shiftwork schedules. Clocks Sleep. (2019) 1:394–413. doi: 10.3390/clockssleep1030032
113. Rosenthal SL, Vakili MM, Evans JA, Elliott JA, Gorman MR. Influence of photoperiod and running wheel access on the entrainment of split circadian rhythms in hamsters. BMC Neurosci. (2005) 6:41. doi: 10.1186/1471-2202-6-41
114. Harrison EM, Gorman MR. Rapid adjustment of circadian clocks to simulated travel to time zones across the globe. J Biol Rhythms. (2015) 30:557–62. doi: 10.1177/0748730415598875
115. Noguchi T, Harrison EM, Sun J, May D, Ng A, Welsh DK, et al. Circadian rhythm bifurcation induces flexible phase resetting by reducing circadian amplitude. Eur J Neurosci. (2018) 51:2329–42. doi: 10.1111/ejn.14086
116. Gorman MR, Yellon SM, Lee TM. Temporal reorganization of the suprachiasmatic nuclei in hamsters with split circadian rhythms. J Biol Rhythms. (2001) 16:552–63. doi: 10.1177/074873001129002240
117. Yan L, Silver R, Gorman MR. Reorganization of suprachiasmatic nucleus networks under 24-h LDLD conditions. J Biol Rhythms. (2010) 25:19–27. doi: 10.1177/0748730409352054
118. Watanabe T, Naito E, Nakao N, Tei H, Yoshimura T, Ebihara S. Bimodal clock gene expression in mouse suprachiasmatic nucleus and peripheral tissues under a 7-hour light and 5-hour dark schedule. J Biol Rhythms. (2007) 22:58–68. doi: 10.1177/0748730406295435
119. Harrison EM, Gorman MR. Changing the waveform of circadian rhythms: considerations for shift-work. Front Neurol. (2012) 3:72. doi: 10.3389/fneur.2012.00072
120. Gorman MR, Elliott JA. Exceptional entrainment of circadian activity rhythms with manipulations of rhythm waveform in male syrian hamsters. Yale J Biol Med. (2019) 92:187–99.
121. Meijer JH, Watanabe K, Schaap J, Albus H, Détári L. Light responsiveness of the suprachiasmatic nucleus: long-term multiunit and single-unit recordings in freely moving rats. J Neurosci. (1998) 18:9078–87. doi: 10.1523/JNEUROSCI.18-21-09078.1998
122. Evans JA, Carter SN, Freeman D a, Gorman MR. Dim nighttime illumination alters photoperiodic responses of hamsters through the intergeniculate leaflet and other photic pathways. Neuroscience. (2012) 202:300–8. doi: 10.1016/j.neuroscience.2011.11.037
123. Evans JA, Gorman MR. In synch but not in step: circadian clock circuits regulating plasticity in daily rhythms. Neuroscience. (2016) 320:259–80. doi: 10.1016/j.neuroscience.2016.01.072
124. Chiesa JJ, Anglès-Pujolràs M, Díez-Noguera A, Cambras T. History-dependent changes in entrainment of the activity rhythm in the Syrian hamster (Mesocricetus auratus). J Biol Rhythms. (2006) 21:45–57. doi: 10.1177/0748730405283654
125. Foster RG, Hughes S, Peirson SN. Circadian photoentrainment in mice and humans. Biology (Basel). (2020) 9:180. doi: 10.3390/biology9070180
126. Mure LS, Le HD, Benegiamo G, Chang MW, Rios L, Jillani N, et al. Diurnal transcriptome atlas of a primate across major neural and peripheral tissues. Science. (2018) 359:eaao0318. doi: 10.1126/science.aao0318
127. Sadun AA, Schaechter JD, Smith LEH. A retinohypothalamic pathway in man: light mediation of circadian rhythms. Brain Res. (1984) 302:371–7. doi: 10.1016/0006-8993(84)90252-X
128. McGlashan EM, Poudel GR, Vidafar P, Drummond SPA, Cain SW. Imaging individual differences in the response of the human suprachiasmatic area to light. Front Neurol. (2018) 9:1022. doi: 10.3389/fneur.2018.01022
129. Li JZ, Bunney BG, Meng F, Hagenauer MH, Walsh DM, Vawter MP, et al. Circadian patterns of gene expression in the human brain and disruption in major depressive disorder. Proc Natl Acad Sci. (2013) 110:9950–5. doi: 10.1073/pnas.1305814110
130. Hofman MA, Zhou J-N, Swaab DF. No evidence for a diurnal vasoactive intestinal polypeptide (VIP) rhythm in the human suprachiasmatic nucleus. Brain Res. (1996) 722:78–82. doi: 10.1016/0006-8993(96)00180-1
131. Yan L, Smale L, Nunez AA. Circadian and photic modulation of daily rhythms in diurnal mammals. Eur J Neurosci. (2020) 51:551–66. doi: 10.1111/ejn.14172
132. Duffy JF, Wright KP. Entrainment of the human circadian system by light. J Biol Rhythms. (2005) 20:326–38. doi: 10.1177/0748730405277983
133. Smale L, Lee T, Nunez AA. Mammalian diurnality: some facts and gaps. J Biol Rhythms. (2003) 18:356–66. doi: 10.1177/0748730403256651
134. Panda S, Hogenesch JB, Kay SA. Circadian rhythms from flies to human. Nature. (2002) 417:329–35. doi: 10.1038/417329a
135. Vansteensel MJ, Michel S, Meijer JH. Organization of cell and tissue circadian pacemakers: a comparison among species. Brain Res Rev. (2008) 58:18–47. doi: 10.1016/j.brainresrev.2007.10.009
136. Peichl L. Diversity of mammalian photoreceptor properties: adaptations to habitat and lifestyle? Anat Rec Part A Discov Mol Cell Evol Biol. (2005) 287A:1001–12. doi: 10.1002/ar.a.20262
137. Roorda A, Williams DR. The arrangement of the three cone classes in the living human eye. Nature. (1999) 397:520–2. doi: 10.1038/17383
138. Calderone JB, Jacobs GH. Regional variations in the relative sensitivity to UV light in the mouse retina. Vis Neurosci. (1995) 12:463–8. doi: 10.1017/S0952523800008361
139. Perry VH, Cowey A. The ganglion cell and cone distributions in the monkey's retina: implications for central magnification factors. Vision Res. (1985) 25:1795–810. doi: 10.1016/0042-6989(85)90004-5
140. Jeon C-J, Strettoi E, Masland RH. The major cell populations of the mouse retina. J Neurosci. (1998) 18:8936–46. doi: 10.1523/JNEUROSCI.18-21-08936.1998
141. Zele AJ, Cao D. Vision under mesopic and scotopic illumination. Front Psychol. (2015) 5:1594. doi: 10.3389/fpsyg.2014.01594
142. Altimus CM, Guler AD, Villa KL, McNeill DS, LeGates TA, Hattar S, et al. Rods-cones and melanopsin detect light and dark to modulate sleep independent of image formation. Proc Natl Acad Sci. (2008) 105:19998–20003. doi: 10.1073/pnas.0808312105
143. Panda S, Sato TK, Castrucci AM, Rollag MD, DeGrip WJ, Hogenesch JB, et al. Melanopsin (Opn4) requirement for normal light-induced circadian phase shifting. Science. (2002) 298:2213–6. doi: 10.1126/science.1076848
144. Ruby NF, Brennan TJ, Xie X, Cao V, Franken P, Heller HC, et al. Role of melanopsin in circadian responses to light. Science. (2002) 298:2211–3. doi: 10.1126/science.1076701
145. Provencio I, Rodriguez IR, Jiang G, Hayes WP, Moreira EF, Rollag MD. A novel human opsin in the inner retina. J Neurosci. (2000) 20:600–5. doi: 10.1523/JNEUROSCI.20-02-00600.2000
146. Berson DM, Dunn FA, Takao M. Phototransduction by retinal ganglion cells that set the circadian clock. Science. (2002) 295:1070–3. doi: 10.1126/science.1067262
147. Hattar S, Liao HW, Takao M, Berson DM, Yau KW. Melanopsin-containing retinal ganglion cells: architecture, projections, and intrinsic photosensitivity. Science. (2002) 295:1065–70. doi: 10.1126/science.1069609
148. Graham DM, Wong KY. Melanopsin-expressing, Intrinsically Photosensitive Retinal Ganglion Cells (ipRGCs). Salt Lake City, UT: The Organization of the Retina and Visual System (1995).
149. Brown TM, Tsujimura S, Allen AE, Wynne J, Bedford R, Vickery G, et al. Melanopsin-based brightness discrimination in mice and humans. Curr Biol. (2012) 22:1134–41. doi: 10.1016/j.cub.2012.04.039
150. Mure LS, Vinberg F, Hanneken A, Panda S. Functional diversity of human intrinsically photosensitive retinal ganglion cells. Science. (2019) 366:1251–5. doi: 10.1126/science.aaz0898
151. Wong KY, Dunn FA, Graham DM, Berson DM. Synaptic influences on rat ganglion-cell photoreceptors. J Physiol. (2007) 582:279–96. doi: 10.1113/jphysiol.2007.133751
152. Dacey DM, Liao H-W, Peterson BB, Robinson FR, Smith VC, Pokorny J, et al. Melanopsin-expressing ganglion cells in primate retina signal colour and irradiance and project to the LGN. Nature. (2005) 433:749–54. doi: 10.1038/nature03387
153. Huberman AD, Niell CM. What can mice tell us about how vision works? Trends Neurosci. (2011) 34:464–73. doi: 10.1016/j.tins.2011.07.002
154. Kolb H. Facts and Figures Concerning the Human Retina. Salt Lake City, UT: The Organization of the Retina and Visual System (1995).
155. Hut RA, van Oort BEH, Daan S. Natural entrainment without dawn and dusk: the case of the european ground squirrel (Spermophilus citellus). J Biol Rhythms. (1999) 14:290–9. doi: 10.1177/074873099129000704
156. DeCoursey PJ. Light-sampling behavior in photoentrainment of a rodent circadian rhythm. J Comp Physiol A. (1986) 159:161–9. doi: 10.1007/BF00612299
157. Harrison EM, Gorman MR, Mednick SC. The effect of narrowband 500 nm light on daytime sleep in humans. Physiol Behav. (2011) 103:197–202. doi: 10.1016/j.physbeh.2011.01.020
158. Cole RJ, Smith JS, Alcalá YC, Elliott JA, Kripke DF. Bright-light mask treatment of delayed sleep phase syndrome. J Biol Rhythms. (2002) 17:89–101. doi: 10.1177/074873002129002366
159. Zeitzer JM, Fisicaro RA, Ruby NF, Heller HC. Millisecond flashes of light phase delay the human circadian clock during sleep. J Biol Rhythms. (2014) 29:370–6. doi: 10.1177/0748730414546532
160. Robinson J, Bayliss SC, Fielder AR. Transmission of light across the adult and neonatal eyelid in vivo. Vision Res. (1991) 31:1837–40. doi: 10.1016/0042-6989(91)90031-Y
161. Ando K, Kripke DF. Light attenuation by the human eyelid. Biol Psychiatry. (1996) 39:22–5. doi: 10.1016/0006-3223(95)00109-3
162. Grubisic M, Haim A, Bhusal P, Dominoni DM, Gabriel KMA, Jechow A, et al. Light pollution, circadian photoreception, and melatonin in vertebrates. Sustainability. (2019) 11:6400. doi: 10.3390/su11226400
163. Brainard GC, Richardson BA, King TS, Matthews SA, Reiter RJ. The suppression of pineal melatonin content and N-acetyltransferase activity by different light irradiances in the Syrian hamster: a dose-response relationship. Endocrinology. (1983) 113:293–6. doi: 10.1210/endo-113-1-293
164. Brainard GC, Hanifin JP, Greeson JM, Byrne B, Glickman G, Gerner E, et al. Action spectrum for melatonin regulation in humans: evidence for a novel circadian photoreceptor. J Neurosci. (2001) 21:6405–12. doi: 10.1523/JNEUROSCI.21-16-06405.2001
165. Brainard GC, Hanifin JP, Warfield B, Stone MK, James ME, Ayers M, et al. Short-wavelength enrichment of polychromatic light enhances human melatonin suppression potency. J Pineal Res. (2015) 58:352–61. doi: 10.1111/jpi.12221
166. Cajochen C, Zeitzer JM, Czeisler CA, Dijk D-J. Dose-response relationship for light intensity and ocular and electroencephalographic correlates of human alertness. Behav Brain Res. (2000) 115:75–83. doi: 10.1016/S0166-4328(00)00236-9
167. Thapan K, Arendt J, Skene DJ. An action spectrum for melatonin suppression: evidence for a novel non-rod, non-cone photoreceptor system in humans. J Physiol. (2001) 535:261–7. doi: 10.1111/j.1469-7793.2001.t01-1-00261.x
168. West KE, Jablonski MR, Warfield B, Cecil KS, James M, Ayers MA, et al. Blue light from light-emitting diodes elicits a dose-dependent suppression of melatonin in humans. J Appl Physiol. (2011) 110:619–26. doi: 10.1152/japplphysiol.01413.2009
169. Chang A-M, Scheer FAJL, Czeisler CA. The human circadian system adapts to prior photic history. J Physiol. (2011) 589:1095–102. doi: 10.1113/jphysiol.2010.201194
170. Hut RA, Oklejewicz M, Rieux C, Cooper HM. Photic sensitivity ranges of hamster pupillary and circadian phase responses do not overlap. J Biol Rhythms. (2008) 23:37–48. doi: 10.1177/0748730407311851
171. Zeitzer JM. When is a proxy not a proxy? The foibles of studying non-image forming light. J Physiol. (2018) 596:2029–30. doi: 10.1113/JP276076
172. Najjar RP, Zeitzer JM. Temporal integration of light flashes by the human circadian system. J Clin Invest. (2016) 126:938–47. doi: 10.1172/JCI82306
173. Hanifin JP, Lockley SW, Cecil K, West K, Jablonski M, Warfield B, et al. Randomized trial of polychromatic blue-enriched light for circadian phase shifting, melatonin suppression, and alerting responses. Physiol Behav. (2019) 198:57–66. doi: 10.1016/j.physbeh.2018.10.004
174. Rahman SA, Hilaire MA, Lockley SW. The effects of spectral tuning of evening ambient light on melatonin suppression, alertness and sleep. Physiol Behav. (2017) 177:221–9. doi: 10.1016/j.physbeh.2017.05.002
175. Chang A-M, Scheer FAJL, Czeisler CA, Aeschbach D. Direct effects of light on alertness, vigilance, and the waking electroencephalogram in humans depend on prior light history. Sleep. (2013) 36:1239–46. doi: 10.5665/sleep.2894
176. Hébert M, Martin SK, Lee C, Eastman CI. The effects of prior light history on the suppression of melatonin by light in humans. J Pineal Res. (2002) 33:198–203. doi: 10.1034/j.1600-079X.2002.01885.x
177. Smith KA, Schoen MW, Czeisler CA. Adaptation of human pineal melatonin suppression by recent photic history. J Clin Endocrinol Metab. (2004) 8:3610–4. doi: 10.1210/jc.2003-032100
178. Zeitzer JM, Friedman L, Yesavage JA. Effectiveness of evening phototherapy for insomnia is reduced by bright daytime light exposure. Sleep Med. (2011) 12:805–7. doi: 10.1016/j.sleep.2011.02.005
179. Jasser SA, Hanifin JP, Rollag MD, Brainard GC. Dim light adaptation attenuates acute melatonin suppression in humans. J Biol Rhythms. (2006) 21:394–404. doi: 10.1177/0748730406292391
180. Vetter C, Phillips AJK, Silva A, Lockley SW, Glickman G. >Light Me up? Why, When, and How Much Light We Need. J Biol Rhythms. (2019) 34:573–5. doi: 10.1177/0748730419892111
181. Wright KP, McHill AW, Birks BR, Griffin BR, Rusterholz T, Chinoy ED. Entrainment of the human circadian clock to the natural light-dark cycle. Curr Biol. (2013) 23:1554–8. doi: 10.1016/j.cub.2013.06.039
182. Stothard ER, McHill AW, Depner CM, Birks BR, Moehlman TM, Ritchie HK, et al. Circadian entrainment to the natural light-dark cycle across seasons and the weekend. Curr Biol. (2017) 27:508–13. doi: 10.1016/j.cub.2016.12.041
183. Prayag AS, Najjar RP, Gronfier C. Melatonin suppression is exquisitely sensitive to light and primarily driven by melanopsin in humans. J Pineal Res. (2019) 66:e12562. doi: 10.1111/jpi.12562
184. Brown TM. Melanopic illuminance defines the magnitude of human circadian light responses under a wide range of conditions. J Pineal Res. (2020) 69:12655. doi: 10.1111/jpi.12655
185. Spitschan M. Melanopsin contributions to non-visual and visual function. Curr Opin Behav Sci. (2019) 30:67–72. doi: 10.1016/j.cobeha.2019.06.004
186. Spitschan M, Stefani O, Blattner P, Gronfier C, Lockley S, Lucas R. How to report light exposure in human chronobiology and sleep research experiments. Clocks Sleep. (2019) 1:280–9. doi: 10.3390/clockssleep1030024
187. Fluxometer. Available online at: https://fluxometer.com/rainbow/
Keywords: entrainment, physiology, translation, parametric effects, circadian, dim light, nLAN
Citation: Walbeek TJ, Harrison EM, Gorman MR and Glickman GL (2021) Naturalistic Intensities of Light at Night: A Review of the Potent Effects of Very Dim Light on Circadian Responses and Considerations for Translational Research. Front. Neurol. 12:625334. doi: 10.3389/fneur.2021.625334
Received: 02 November 2020; Accepted: 06 January 2021;
Published: 01 February 2021.
Edited by:
Manuel Spitschan, University of Oxford, United KingdomReviewed by:
Randy J. Nelson, West Virginia University, United StatesStuart N. Peirson, University of Oxford, United Kingdom
Travis Longcore, UCLA Institute of the Environment and Sustainability, United States
Copyright © 2021 Walbeek, Harrison, Gorman and Glickman. This is an open-access article distributed under the terms of the Creative Commons Attribution License (CC BY). The use, distribution or reproduction in other forums is permitted, provided the original author(s) and the copyright owner(s) are credited and that the original publication in this journal is cited, in accordance with accepted academic practice. No use, distribution or reproduction is permitted which does not comply with these terms.
*Correspondence: Michael R. Gorman, mgorman@ucsd.edu
†ORCID: Thijs J. Walbeek orcid.org/0000-0002-2907-2126
Elizabeth M. Harrison orcid.org/0000-0002-0482-589X
Michael R. Gorman orcid.org/0000-0001-6122-8437
Gena L. Glickman orcid.org/0000-0001-7064-7238
‡These authors have contributed equally to this work and share first authorship